UNC Scientists Design 3D Cathodes to Make CO2-to-Fuel Conversion More Efficient
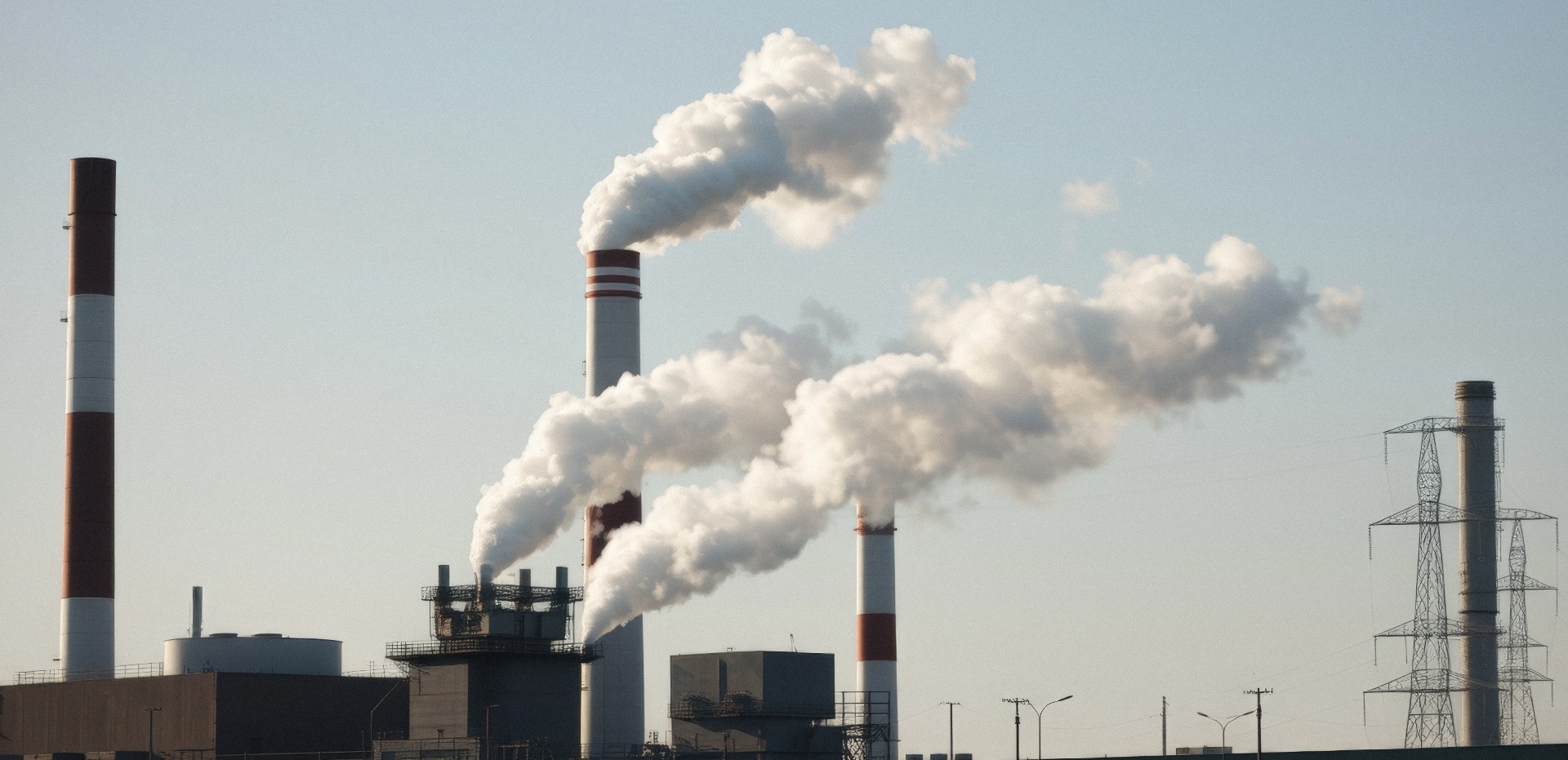
Instead of allowing carbon dioxide to accumulate in the atmosphere, UNC scientists are investigating methods to recycle it into useful products.
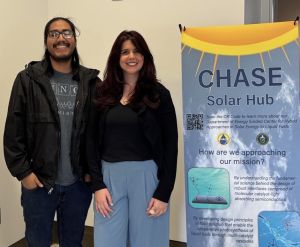
March 28, 2025 I By Dave DeFusco
Imagine a world where we could capture excess carbon dioxide (CO2) from the atmosphere and transform it into fuel like methanol. This concept excites scientists because it presents a dual solution: reducing greenhouse gases while providing a sustainable energy source. However, the conversion of CO2 into fuel is fraught with challenges—chemical reactions are often slow, inefficient and produce unwanted byproducts.
Supported by the Center for Hybrid Approaches in Solar Energy (CHASE) to Liquid Fuels, researchers at UNC-Chapel Hill led by Dr. Rene Lopez, a professor in Applied Physical Sciences and in Physics and Astronomy, have taken a significant step toward overcoming these obstacles. Their ACS Catalysis study, “Spatially Patterned Architectures to Modulate CO2 Reduction Cascade Catalysis Kinetics,” explores how structuring catalysts in a precise pattern can make the process more efficient.
Carbon dioxide is a leading contributor to climate change. Instead of allowing it to accumulate in the atmosphere, scientists are investigating methods to recycle it into useful products. One promising approach is electrochemical CO2 reduction, which uses electricity from renewable sources to break down CO2 and convert it into fuels; however, this process is inherently complex due to the interactions between chemical reactions and molecular diffusion at the electrode surface.
Catalysts are essential in chemical reactions, as they speed up the transformation of CO2 into valuable products through a step-by-step process known as a cascade reaction. Typically, catalysts are placed on flat electrode surfaces, but this traditional approach can result in inefficiencies. Intermediate chemicals produced in one step often do not reach the next catalyst in the sequence, leading to inefficiencies and unwanted side reactions.
“We hypothesized that by structuring the catalyst environment in a three-dimensional trench-like pattern, they could improve the efficiency of the CO2 reduction reaction,” said Dr. Marisé García-Batlle, a lead author of the study and postdoctoral research associate at UNC. “To test our idea, we created a computer model simulating an electrode surface with catalysts embedded in periodic trenches.”
The model allowed them to analyze key factors affecting reaction efficiency:
- Better Mass Transport—The trench design improved the movement of reactants between catalytic sites, enhancing overall efficiency.
- Controlled Local Chemistry—The trench geometry influenced pH levels near the electrode, impacting reaction rates and selectivity.
- Increased Product Yield—The structured design helped produce more desirable fuels, like methanol, while reducing unwanted byproducts like hydrogen gas.
- Optimized Trench Design—The researchers identified the ideal trench depth and arrangement for maximizing efficiency, discovering that past a certain depth, benefits plateaued.
By containing the intermediate chemicals in a controlled environment, the trench design ensured they efficiently reached the next catalyst in the sequence, reducing the likelihood of side reactions and improving the overall process.
“One of the key discoveries in the study was how the trench design influences local pH levels, which play a crucial role in reaction selectivity,” said Pablo Fernandez, the other lead author and a Ph.D. student in the UNC Department of Chemistry. “In traditional catalyst setups, reactants may not consistently interact with the catalyst surface, reducing efficiency; however, in the trench design, molecules are forced to collide with the catalyst surfaces, leading to more frequent and effective reactions.”
The confined space within the trenches also causes localized changes in pH. As pH increases in these microenvironments, the reaction is steered toward producing more of the desired fuel product, such as methanol, while minimizing the formation of unwanted side products like hydrogen gas. However, there are limits to this effect. If pH gets too high, carbon dioxide availability decreases because bicarbonate ions begin to form, reducing the efficiency of the overall cascade reaction.
“We suggest adjusting solvents or buffer concentrations as a strategy to stabilize carbon dioxide levels and prevent unwanted side reactions,” said Fernandez.
While the study primarily focused on trench geometries, the researchers are also exploring other three-dimensional structures, such as micropillars, which could further improve reaction efficiency. These alternative structures may offer even better control over mass transport and reaction selectivity, opening new avenues for optimizing CO2 conversion technologies.
The team is now looking for catalysts capable of performing cascade reactions effectively. One potential candidate is cobalt phthalocyanine p, a material that has shown promise in facilitating the necessary reaction steps. Their model provides a theoretical foundation for testing different catalysts and refining electrode designs in future experiments.
“While this research is still in the theoretical stage, it represents a significant step forward in designing more efficient carbon dioxide reduction systems,” said Dr. Lopez, who is senior author of the paper. “By optimizing electrode geometry and improving catalyst placement, we can develop scalable technologies to convert carbon dioxide into useful fuels, transforming a harmful waste product into a valuable resource.”